In the world of imaging technologies, there’s a constant quest for higher sensitivity, faster acquisition, and better signal-to-noise ratios. Three notable contenders in this pursuit are Electron Multiplying Charge-Coupled Device (EMCCD), scientific Complementary Metal-Oxide-Semiconductor (sCMOS), and Single-Photon Avalanche Diode (SPAD) detectors. Each comes with its own set of advantages and limitations, catering to diverse applications in scientific research, medical imaging, and beyond.
EMCCD (Electron Multiplying Charge-Coupled Device)
EMCCD cameras have been the “go-to” camera for photon starved applications for decades. The key to their sensitivity is in the electron multiplication stage: as the photo-electrons are read off the sensor, they pass through the multiplication register. At each stage of this process electrons are subjected to voltage potential that creates more photo-electrons through the process of impact ionization. The probability of an additional electron being produced is typically between 1% and 1.6% for each gate, but since the number of gates in the multiplication register is on generally on the order of 500 gates, amplification gains of up to 1000x are possible.
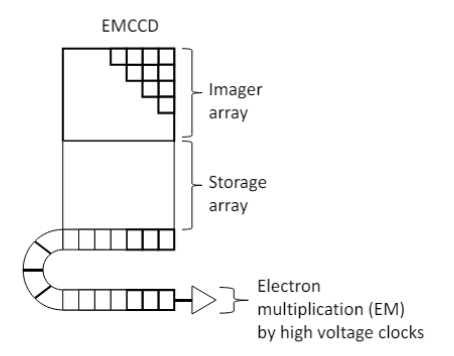
Key Points for EMCCDs:
- Very Low Read Noise: a single photoelectron amplified 1000x makes the relative read noise insignificant
- Excess Noise Factor: the probabilistic nature of the gain introduces a noise component not seen in CMOS or CCD cameras, this value has a theoretical maximum of 2.
- Keep it Cold: the multiplicative gain varies significantly with temperature, so these cameras are typically cooled to -70C or colder.
- Clock-induced Charge: spurious electrons can be produced during any gate transfer. In a conventional CCD the value is so low as to be insignificant, but with an EMCCD these spurious electrons can be multiplied, introducing one more noise factor not present with other cameras.
- Limited Dynamic Range: with a single photon becoming 1000 photoelectrons, the well capacity can quickly fill up, limiting the dynamic range of the camera.
Advantages of EMCCD
- High Sensitivity: EMCCDs excel at detecting weak signals, making them ideal for low-light conditions.
- Low Noise: The on-chip electron multiplication minimizes readout noise, enhancing signal.
- Single-Photon Detection: Some EMCCD models can detect single photons.
Limitations of EMCCDs:
- Limited Dynamic Range: EMCCDs may struggle with scenes containing both bright and dim regions due to their restricted dynamic range.
- Quantitative Measurements: Due the probabilistic nature of the Multiplication register on an EMCCD not all electrons are amplified equally.
sCMOS (scientific Complementary Metal-Oxide-Semiconductor):
sCMOS cameras have emerged as powerful tools in various imaging applications, offering a balance between sensitivity, speed, and resolution.
Enormous improvements in uniformity, noise reduction and quantum efficiency (QE) over the past decade have made the sCMOS the camera of choice in many applications. Unlike EMCCDs, each pixel on an sCMOS detector has its own amplifier converting photons to electronic signal on the spot. The back-thinning, or back-illumination of sCMOS sensors has improved the QE, by having the electronics for each pixel on the side opposite that of the incident light: electronics on the ‘front’ and photons incident on the ‘back. Lines of pixels share an analog-to-digital converter (ADC). This design allows for rolling shutter capability and makes them well-suited for high-speed imaging tasks, including live-cell microscopy and particle tracking.

QE: Quantum Efficiency is the percentage of photons detected at a given wavelength. Peak QEs for back-illuminated sensors are upwards of 95%
Key Points for sCMOS:
- Back-illuminated designs have pushed the QE to over 90%
- Improvements to readout amplifiers working in ultra-quiet modes have reduced the read noise to 1 e- or less in some cameras.
- Non-uniform amplification was once an issue as each pixel has its own amplification electronics, but this is no longer an issue. Improved production quality has made this problem non-existent for high-end sCMOS cameras.
Advantages of sCMOS:
- High Speed: sCMOS cameras can capture rapid events with high frame rates, facilitating dynamic imaging studies.
- Large Dynamic Range: These detectors offer a broad dynamic range, accommodating both bright and dim signals within the same image.
- Large Format: because of the architecture of the sCMOS, it is possible to make large sensors with no penalty aside from reading off large amounts of data.
- Cost-Effectiveness: sCMOS cameras are offered in a very wide range of cameras and at a price point for every user. The ubiquitousness of these sensors has meant these high-quality cameras are more affordable than ever.
Limitations of sCMOS:
- Noise: With sCMOS cameras there is a trade-off between speed and noise. Many manufacturers now offer ultra-low noise readout modes, but these are associated with slower frame rates. Some sCMOS have read noise less than 1e-
- Photon Counting Limitations: With very few exceptions, sCMOS detectors are not suitable for single-photon detection applications due to their intrinsic noise characteristics.
SPAD (Single-Photon Avalanche Diode)
SPAD detectors represent the pinnacle of sensitivity, capable of detecting individual photons with remarkable efficiency. These devices leverage the phenomenon of avalanche multiplication within a semiconductor structure, allowing them to detect single photons. SPAD technology finds applications in fields such as quantum imaging, LIDAR, and fluorescence lifetime imaging microscopy (FLIM). These cameras are fundamentally different from CCD or CMOS technology. CCD or CMOS accumulate signal from detected photons during a given exposure time (up to the well capacity of the pixel) whereas the SPAD is essentially a digital device, triggered once it detects a photon. A SPAD operates in a modewhat is called “Geiger mode” where a single incoming photon will create an electron-hole pair which will be amplified enough to create a measurable current. SPADs are intrinsically able to detect single-photons, with a very high temporal resolution. Images with a bit-depth greater than one are created by aggregating frames to build up the desired bit-depth. For example, 16 frames are required to create a 4-bit image (24=16).

Key Points for SPADs:
- Single Photon Sensitivity: With low dark current and zero read noise, a signal is almost always from the detection of a single photon.
- Fast Acquisition: For 1-bit images, acquisition rates can be 100,000 frames per second.
- Gating Capabilities: gates as small as 6ns with picosecond resolution are possible.
Advantages of SPADs:
- Single-Photon Sensitivity: SPAD detectors can detect individual photons, enabling exquisite sensitivity in low-light environments.
- High Speed: SPADs offer rapid response times, making them suitable for high-speed imaging and photon counting applications.
- Zero Read Noise: With a binary detection of photons, the read noise is zero
- Gating capability: combined with high speed and single-photon sensitivity being able to gate the acquisition give them capabilities on par with Intensified Imagers.
- Quantum Imaging: SPADs play a crucial role in quantum imaging setups, where single-photon detection is essential for capturing quantum phenomena.
Limitations of SPADs:
- Limited Dynamic Range: SPAD detectors collect binary frames and build up bit depth through accumulation of multiple frames. While they, theoretically, have no limit to the Dynamic Range (one can keep acquiring more frames), this impacts the overall speed of acquisition. To capture a 16-bit image would result in an acquisition rate of 1/(2 16); for a 1-bit frame rate of 100k fps that would lead to
just over 1.5 fps. - Spatial Resolution: to date the pixels on SPAD arrays are larger than those for EMCCDs and sCMOS
- Quantum Efficiency: The electronics needed to create a SPAD are such that the active area, that which detects the photon, is only a fraction of the area of each pixel. This is addressed by adding lenses on each pixel to direct light to the active area.
Comparing Technologies:
Comparing two sCMOS cameras can be complicated with all the different readout settings and different pixel sizes on all the different cameras. Comparing different devices is even more difficult.
Signal-to-Noise (SNR) is defined as the total number of photons detected divided by all the noise in the system. As discussed above the different sensors have different noise sources: for example, the EMCCDs have Clock-Induced-Charge (CIC) whereas the SPAD has zero read noise, due to its inherent properties.
The graph below assumes that all the detectors have the same size pixel. There is a plethora of different detectors with different specifications. To get an idea of how these different technologies compare the following values were used for the theoretical calculations:
EMCCD | sCMOS | SPAD | |
---|---|---|---|
QE / QEeff | 0.9 | 0.95 | 0.45 |
Excess Noise (E) | 1.414 | 1 | 1 |
Dark Noise (B) | 0.00000002 | 0.000024 | 0.002 |
Dark Current (e/p/s) | 0.00025 | 0.3 | 25 |
Exp Time (s) | 0.00008 | 0.00008 | 0.00008 |
Readout Noise (R) | 0.1 | 0.27 | 0 |
Multiplication Gain (M) | 1000 | 1 | 1 |
No. of Frames (F): for SPAD | 4016 (12-bit) | ||
Spurious Events e/px @1000x | 0.005 | ||
Pixel Size | 10 | 10 | 10 |
Note the Dark Noise was calculated by multiplying the Dark Current by the Exposure time. The short exposure time was chosen as that is typical for a SPAD array taking a 12-bit image.
As the number of photons per pixel increases the sCMOS gets close to the ideal detector.
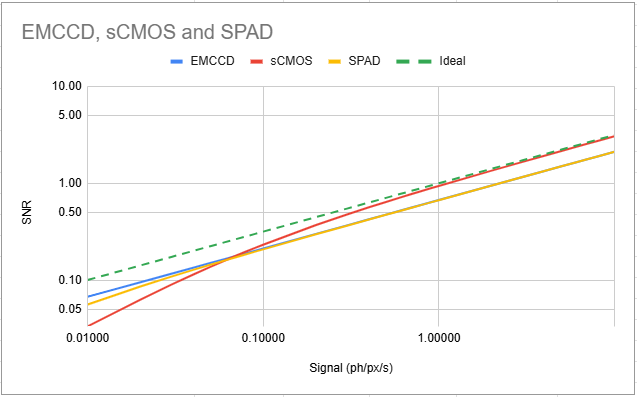
At low photon flux, as expected, the EMCCD and the SPAD detectors outperform the sCMOS. The EMCCD has a slightly better SNR at very low light levels.
Looking at the theoretical SNR may not suffice. The fact that the SPAD camera acts as a true photon to digital converter gives it a superior photon detection fidelity. Pi Imaging created the following comparison:
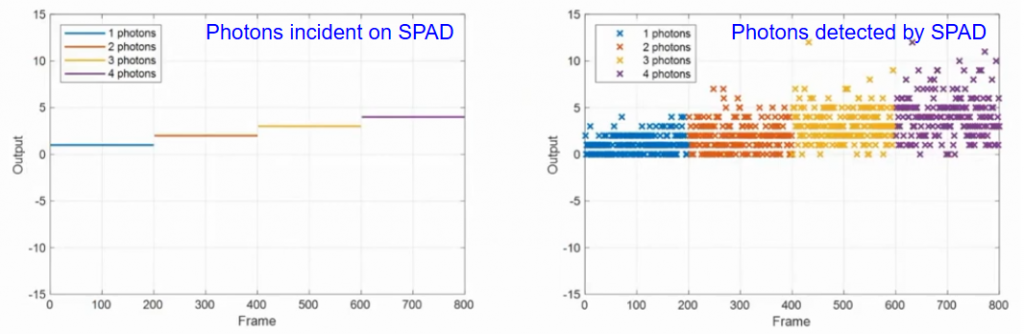
In this experiment, 800 frames are captured. For the first 200 frames, a single photon is incident on the detector for each frame; for the second 200 frames, two photons are incident on the detector per frame, 3 photons for the next 200 frames and then 4 photons for the last 200 frames. The SPAD detector is not perfect and it detects the randomness associated with the quantum nature of light. If this data is represented as a histogram of detection over the entire collection of 800 frames, then the digital nature of the SPAD detector is apparent:
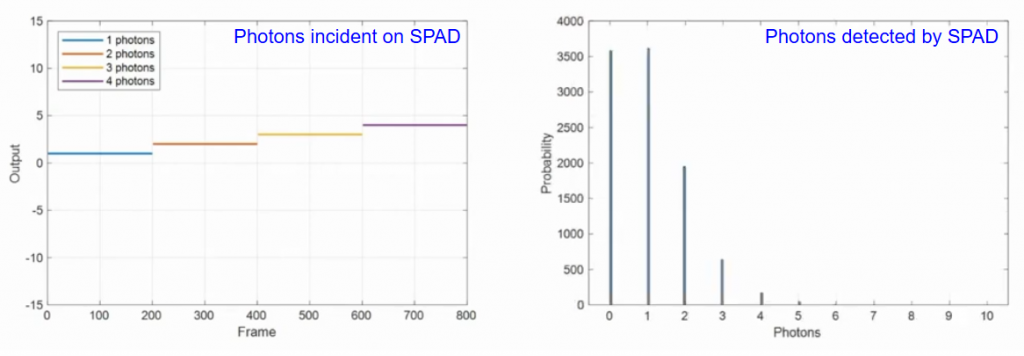
Repeating this exercise for both sCMOS and EMCCD gives very different distributions due to the noise characteristics and the probabilistic nature of the EMCCD gain. To get photon counting one must back calculate the signal based on the gain settings of the camera – this can give non-integer values for the number of photons detected.
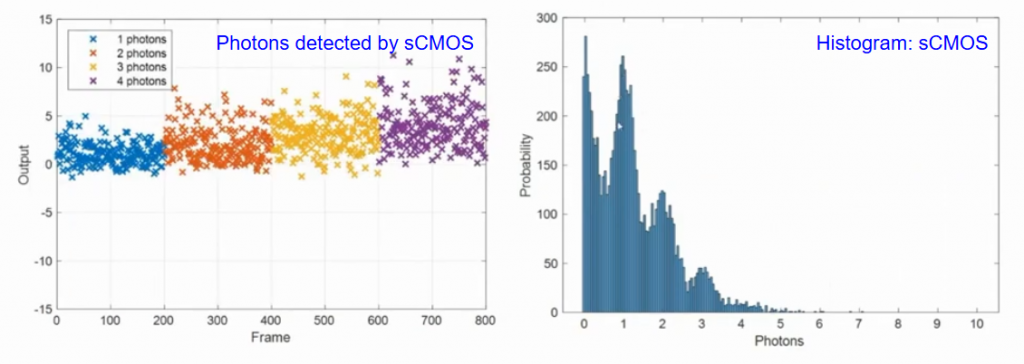
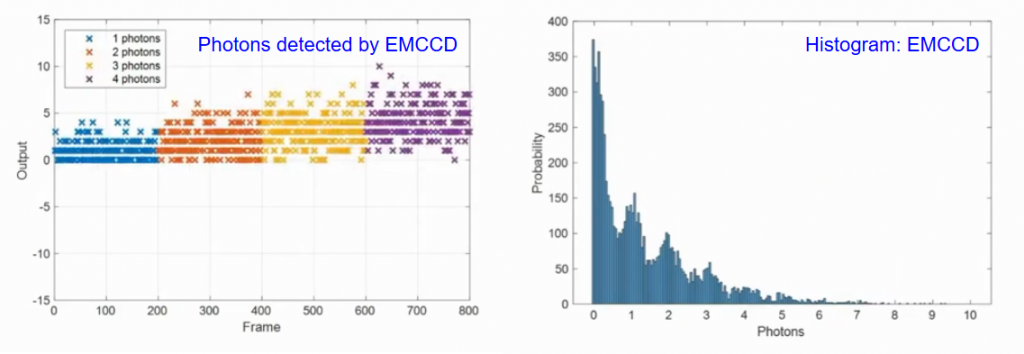
The distribution for sCMOS has clearer definitions of when a single photon is detected versus the EMCCD, but neither comes close to the SPAD for fidelity of single photon detection.
Conclusion
In the realm of advanced imaging technologies, EMCCD, sCMOS, and SPAD detectors each offer unique capabilities suited to specific applications. EMCCDs excel in sensitivity, making them ideal for low-light imaging tasks, the combination of bit-depth, speed and sensitivity is amazing. The sCMOS cameras prioritize speed and versatility; detectors come in many shapes and sizes, QE is upward of 95% for high-end detectors and they are. SPAD detectors, on the other hand, push the boundaries of sensitivity and speed, enabling groundbreaking research in quantum imaging and photonics. When single photon detection is needed, bit-depth is not important, speed and sensitivity are. This is where the SPAD detector is truly a superior detector. Furthermore, the SPAD detectors are capable of operating as gated devices, not possible with sCMOS or EMCCD. Understanding the strengths and limitations of each technology is essential for selecting the most suitable solution for a given imaging challenge.
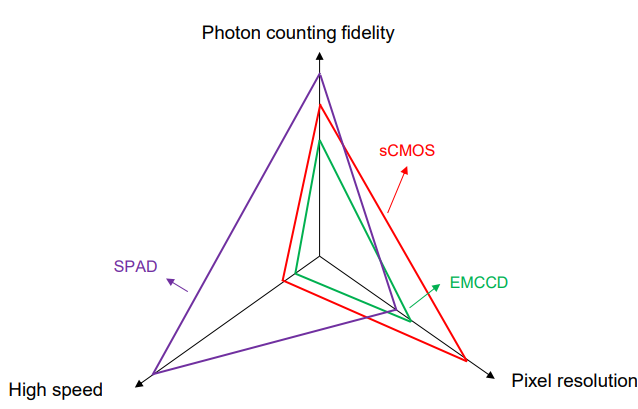